Understanding impacts of atmospheric oxidation processes in the remote marine boundary layer on greenhouse gases
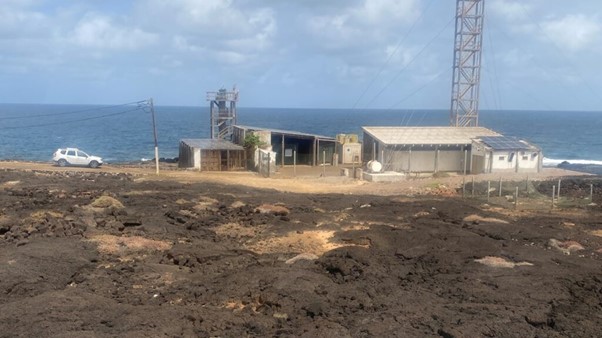
The studentship will remain open until a suitable applicant is found.
Please note: Applications from international (non-home fee rated) candidates cannot be accepted after 30 May 2025
Roughly a quarter of tropospheric methane removal (a key greenhouse gas, GHG) occurs in the tropical marine boundary layer (MBL) driven by the reaction with the hydroxyl radical, OH (Bloss et al., 2005). With a knowledge of OH sources and OH sinks, atmospheric OH concentrations can be modelled and compared to observations. Using this approach, recent observations and model comparisons have highlighted a number of key uncertainties in our understanding of the chemical processing occurring in the remote MBL, which limits our understanding of the role atmospheric chemistry plays in controlling climate.
Recent MBL observations of OH reactivity, kOH, which is a measure of the total OH loss rate, have demonstrated that there are unknown species which contribute to OH destruction in these environments (Thames et al., 2020). This missing or unknown kOH implies that our understanding of the quantity of MBL volatile organic compound (VOC) emissions and the impact these emissions have on OH (and, therefore, methane lifetime) is incomplete. Missing kOH hinders our ability to accurately predict peroxy radical concentrations (formed from VOC oxidation by OH) which can lead to the formation of another important GHG, ozone, or the formation of secondary organic aerosol (SOA) which influence cloud formation and the earth’s radiative balance.
Field observations and modelling studies have also demonstrated that halogen species in the MBL influence the budgets of ozone (O3), HOx (HOx = OH + HO2), and NOx (NOx = NO + NO2), as well as aerosol formation. The production of bromine and iodine atoms in the MBL following emissions of organohalogen compounds and inorganic compounds such as Br2, I2, and HOI has been shown to result in considerable destruction of tropospheric ozone (Read et al., 2008), and leads to the production of the radicals bromine monoxide (BrO) and iodine monoxide (IO). In the MBL, halogen monoxides (XO, where X = halogen) react with hydroperoxy radicals (HO2) to produce hypohalaous acids (HOX) which photolyse during the day, releasing hydroxyl radicals (OH) and halogen atoms (X):
XO + HO2 → HOX + O2
HOX + hν → OH + X
The chemistry of halogen monoxides thus shifts the balance between OH and HO2 and impacts the lifetime of CH4 and other GHGs, which are primarily removed from the atmosphere by reaction with OH. The impacts of halogen species on OH and HO2 have been demonstrated in a number of field and modelling studies (e.g. Whalley et al., 2010; Stone et al., 2018). However, recent measurements and modelling of radical species by the Leeds group in the MBL at the Cabo Verde Atmospheric Observatory (CVAO), which included the first simultaneous measurements of OH, HO2, organic peroxy radicals (RO2) and OH reactivity in the MBL, have indicated the potential importance of the chemistry between halogen monoxides and organic peroxy radicals (RO2). Our understanding and ability to assess the atmospheric impacts of XO + RO2 chemistry, however, is hindered by uncertainties in reaction kinetics and product yields, particularly for IO + CH3O2:
IO + CH3O2 → products
An understanding of both the total OH sinks and how halogen chemistry mediates atmospheric oxidation in the MBL is critical for the accuracy and reliability of atmospheric models used to assess and predict the impacts of our changing atmosphere on climate.
This PhD project will address these key uncertainties surrounding MBL chemistry using a combination of field observations, modelling and laboratory experiments. You will take part in a large field project (Closing the budget in marine atmospheric Oxidative Capacity through the quantification of Oceanic VOC emissions, COCO-VOC). As part of COCO-VOC, a comprehensive suite of VOC observations, alongside a measurement of kOH will be made 1) from the island archipelago of Cabo Verde (Figure 1, located in the tropical Atlantic ocean) and 2) on a research ship cruise, which will permit observations over wider spatial scales, including in and around the Mauritanian upwelling which stimulates enhanced biological activity (and VOC production).
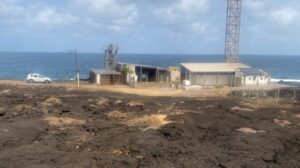
The total OH loss rate is measured using the laser flash-photolysis, laser-induced fluorescence (LFP-LIF) (Stone et al., 2016) method, and this technique has been used successfully by the Leeds team on numerous field campaigns (e.g. Whalley et al., 2016, 2021; Woodward-Massey et al., 2023) including recent observations at Cabo Verde in February 2023.
The successful candidate will also perform a detailed and comprehensive laboratory study of the kinetics and product yields of reactions between halogen monoxides and organic peroxy radicals, with a focus on IO + CH3O2. You will use laser flash photolysis coupled with broadband time-resolved UV absorption spectroscopy (Figure 2, Lewis et al., 2018; Mir et al., 2020; Lade et al., 2024) to determine reaction kinetics over a range of atmospherically relevant conditions, including temperature, which will enable the simultaneous measurement of IO and CH3O2, as well as the potential products CH2OO and OIO.
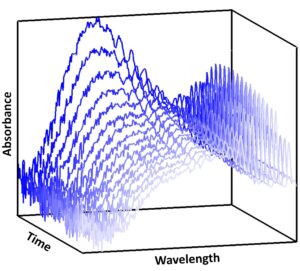
You will also have opportunities to investigate the production of CH3O radicals using laser-induced fluorescence (LIF) spectroscopy (Onel et al., 2017), and of other species using time-resolved mid-infrared quantum cascade laser absorption spectroscopy (Figure 3, Mir et al., 2022).
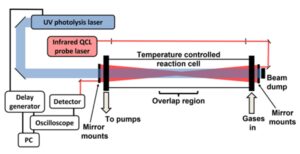
The atmospheric impacts of the experimental results will be determined using atmospheric chemical models based on the Master Chemical Mechanism (MCM) (e.g. Figure 4, Whalley et al., 2010; Stone et al., 2018) and compared to field measurements made during the COCO-VOC project.
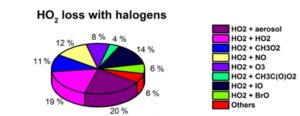
Training
You will work under the supervision of Dr Lisa Whalley and Professor Daniel Stone from the School of Chemistry at Leeds, who are members of the Atmospheric and Planetary Chemistry Group. The supervisors lead active and vibrant research groups exploring the role of gas-phase and aerosol chemical processes in the atmosphere, using experimental and modelling approaches.
You will work in well-equipped laboratories and be part of an active, thriving and well-funded atmospheric chemistry community. The Leeds group has an internationally leading reputation in atmospheric chemistry for field measurements of atmospheric composition, laboratory studies of chemical kinetics and photochemistry, and the development of numerical models and chemical mechanisms. Activities in these three areas are intimately linked and interdependent, providing significant advantages. You will be supported to attend both national and international conferences, and will receive a wide range of training, for example in communication skills, project management, and with other technical aspects. The PhD will provide a wide range of experience in the use of high power lasers, vacuum systems, optics, computer controlled data acquisition systems and methods in numerical modelling. You will also have access to training provided by the National Centre for Atmospheric Science. The successful PhD student will have access to a broad spectrum of training workshops that include managing your degree and preparing for your viva.
Your Profile
You should have an interest in atmospheric chemistry, air quality, climate and global environmental problems, with a strong background in chemistry or a similar discipline (e.g. natural sciences, environmental science, physics, engineering).
References
Bale et al., Physical Chemistry Chemical Physics, 7, 2164-2172, 2005
Bloss et al. Faraday Discussions,130, 425-436, 2005
Dillon et al., Physical Chemistry Chemical Physics, 8, 5185-5198, 2006
Dillon et al., ChemPhysChem, 11, 4011-4018, 2010
Enami et al., Journal of Physical Chemistry A, 110, 9861-9866, 2006
Lade et al., Environmental Science: Atmospheres, 4, 1294-1308, 2024
Lewis et al., Review of Scientific Instruments, 89, 1-8, 2018
Mir et al., Physical Chemistry Chemical Physics, 22, 9448-9459, 2020
Mir et al., Atmospheric Measurement Techniques, 15, 2875-2887, 2022
Onel et al., Atmospheric Measurement Techniques, 10, 3985-4000, 2017
Read et al., Nature, 453, 1232-1235, 2008
Stone et al. Atmospheric Measurement Techniques, 9, 2827-2844, 2016
Stone et al., Atmospheric Chemistry and Physics, 18, 5, 3541-3561, 2018
Thames et al. Atmospheric Chemistry and Physics, 20, 4013-4029, 2020
Whalley et al., Atmospheric Chemistry and Physics, 10, 4, 1555-1576, 2010
Whalley et al. Atmospheric Chemistry and Physics, 16, 2109–2122, 2016
Whalley et al. Atmospheric Chemistry and Physics, 21, 2125–2147, 2021
Woodward-Massey et al. Atmospheric Chemistry and Physics, 23, 14393–14424, 2023